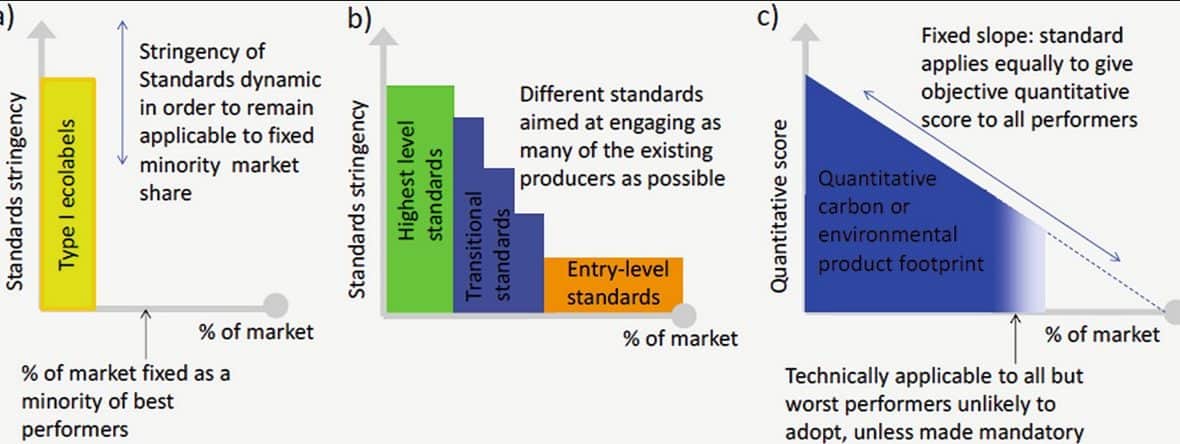
Variations in atmospheric CO2 (measured from air bubbles trapped in ice cores) have generated perhaps the most significant interest regarding the internal mechanisms proposed to explain Pleistocene climate change. The recognition that CO2 variations appeared to correlate extremely well with temperature over the last 160Ka (Barnola et al., 1987; Figure 5.12) suggested that changes in greenhouse forcing was playing a part in Pleistocene climate regulation. During the last major glacial-interglacial transition (at approximately 14Ka), when global temperatures increased by about 4 to 5C, atmospheric CO2 concentration rose 80ppm, from 200ppm to 280ppm (see Figure 5.12).
Hypotheses as to the causes of CO2 changes, and the phase relationships of CO2, ice volume and temperature, have passed through many stages over the last decade or so. All of them involve a redistribution of carbon between its major global reservoirs – atmosphere, ocean, land biosphere and oceanic sediments. (The carbon cycle is fully reviewed in chapter 6, in the context of contemporary climate change.) Most importantly, the time scale of carbon redistribution must match that of the CO2 variations; tectonic processes, which may have accounted for CO2 variations throughout the Phanerozoic (see section 5.2) can therefore be discounted.
Over time scales of millennia, the ocean can be considered to be the most important carbon reservoir, in terms of its potential to influence CO2 changes. Dissolved inorganic carbon in the ocean, or “total CO2” (CO2) includes HCO3– (carbonate ion) (90%), CO32- (bicarbonate ion) (9%) and dissolved CO2 gas (CO2 (aq)) (1%). These agents exist in equilibrium, according to Equation 14.
CO2 (aq) + CO32- + H2O 2HCO3–
[Equation 14]
Atmospheric CO2 is directly regulated by the chemical composition of the surface sea water, according to Equation 15.
CO2 + H2O H2CO3 {CO2 (aq)}
[Equation 15]
Equations 14 and 15 combine to form Equation 16, the governing equation for carbonate chemistry in the ocean system.
CO32- + H2CO3 2HCO3–
[Equation 16]
Equation 15 can be simplified to:
Cw = sCa[Equation 17]
where Cw is the CO2 concentration (or partial pressure) in water, Ca is the CO2 concentration (or partial pressure) in air and s is the solubility (equilibrium) constant for Equation 15.
There are two ways in which the concentration of atmospheric CO2 (Ca) can be altered: varying s or varying Cw. The solubility of CO2 increases with decreasing temperature, thus decreasing Ca for constant Cw. One could postulate that changes in surface ocean temperature associated with glacial-interglacial transitions could indirectly influence the strength of greenhouse forcing; cooler surface oceans during a glacial episode would lower CO2 levels in the atmosphere, thereby initiating a positive feedback. Unfortunately, evidence from the last major glacial-interglacial transition, at about 14Ka, does not support this hypothesis. The magnitude of the global sea surface temperature change (inferred from oxygen isotope records of sediments cores) would only account for about 25% of the change in CO2 (Broecker, 1982). In addition, changes in salinity due to changes in ice volume affect CO2 concentrations in an opposite direction to the effect of temperature fluctuations. Consequently, another mechanism to explain the nature of the CO2 variations is needed.
A 1% increase in CO2 in the surface ocean increases Cw, and consequently Ca by about 10%. Minor changes in the total dissolved inorganic carbon may therefore have considerable effect on atmospheric CO2. CO2 in the surface ocean is smaller than for the entire ocean (Broecker & Peng, 1982). Surface-dwelling plankton (which make up 90% of ocean biota) extract carbon for both photosynthesis and carbonate shell formation. On death, these organisms sink to the sea floor and the carbon is oxidised, returning it to the deep ocean water. Consequently, a CO2 gradient between surface and deep ocean exists (Figure 5.13a).
If the biological productivity of the surface ocean could vary, this would have a direct effect on CO2 in the atmosphere. To explain reduced glacial CO2 levels in the atmosphere, Broecker (1982) suggested that transfer of nutrients from eroding continental shelves, exposed by the marine regression (low sea stand), would raise the productivity level of the ocean, with the increased biomass in the surface waters acting as a “biological pump” to draw down atmospheric CO2. Broecker (1974) argued that since marine plankton preferentially use 12C for photosynthesis, leaving surface water enriched in 13C (see Figure 5.13b), past productivity levels in the ocean could be monitored by measuring the differences in 13C between the surface (13Cplanktonic) and deep (13Cbenthic) waters. Increased surface productivity would increase the difference between surface and deep 13C, 13C (as measured from the carbonate tests of planktonic and benthic organisms respectively).
Unfortunately, changes in 13C over the last 160Ka do not correspond well with variations in atmospheric CO2. In addition, deposition and erosion of shelf sediments is a slow process and depends upon major changes in sea level. Such a response time is too long to account for the more rapid fluctuations in CO2. Finally, evidence of nutrient variations, in particular dissolved phosphate, does not seem to reveal significant changes between glacial and interglacial nutrient content of the oceans (Boyle & Keigwin, 1987).
A number of other factors have been considered which may influence atmospheric CO2 concentrations. Two of the more significant hypotheses are detailed here.
1) Changes in the organic carbon/carbonate ratio of biogenic material falling out of the upper water column (Broecker & Peng, 1982). Increased upwelling in equatorial oceans, due to enhanced glacial trade winds, would raise biogenic productivity. Carbonate-secreting plankton are less common in more productive regions of the ocean, at the expense of siliceous plankton. Thus, enhanced upwelling might cause a shift in the dominant phytoplankton group, which in turn would cause a change in the organic carbon/carbonate rain ratio. With less carbonate (CO32-) falling out of the upper water column, its concentration would increase, thereby raising the alkalinity of the surface waters
. Atmospheric CO2 is very sensitive to changes in alkalinity (Sarmiento et al., 1988); an increase in ocean alkalinity would cause a decrease in pCO2.
2) Enhanced utilisation of nutrients in high-latitude surface waters (Wenk & Siegenthaler, 1985; Toggweiler & Sarmiento, 1985). At present, not all nutrients are consumed in high-latitude oceans because vertical ocean circulation rates are fast enough to prevent equilibrium between phytoplankton fertility and nutrient levels. During the last glacial episode, production rates of the North Atlantic Deep Water (NADW) decreased by as much as 50% (Boyle & Keigwin, 1987), allowing a more efficient nutrient utilisation to take place. Consequently, the glacial North Atlantic was nutrient-depleted whilst deep ocean water became nutrient-enriched (Figure 5.14). The subsequent reduction in surface CO2 would lower pCO2, thereby reducing the atmospheric concentration of CO2. Model calculations show that a 50% reduction in high latitude vertical ocean circulation would cause a 50ppm decrease in atmospheric CO2, almost two-thirds of the observed CO2 change at the last glacial-interglacial transition (Sarmiento & Toggweiler, 1984; Siegenthaler & Wenk, 1984). Possible explanations for the slow down in vertical thermohaline circulation include the larger extent of sea ice, and colder sea surface temperatures (see section 2.6.4).
Again, both these suppositions have their problems. On current evidence, it seems implausible that ocean circulation changes in the small area of the North Atlantic Ocean could account for the entire glacial-interglacial difference in pCO2. Changes in ocean circulation in the Southern Ocean around Antarctica would need to be invoked, but there is no clear evidence for reduced vertical exchange rates during the last glacial period. In addition, enhanced utilisation of surface nutrients, and subsequent increase in high-latitude biogenic productivity, predicts an increase in the 13Cplanktonic. The available isotope data seem not to confirm this expectation.
Despite the obvious difficulties in linking changes in ocean productivity (and ocean circulation and ocean chemistry) with variations in atmospheric CO2, other evidence appears to suggest a definite relationship. Most significantly, an index of non-sea salt (nss) sulphate from an ice core at Vostok, Antarctica (Legrand et al., 1988) correlates well with the ice core deuterium/temperature record (Figure 5.15). Nss sulphates are produced by marine phytoplankton (section 1.3.3); an index of nss sulphate is therefore a measure of surface ocean primary productivity. High levels of nss sulphate coincide with cooler temperatures. Hence, it appears that ocean productivity (and presumably atmospheric CO2) is in some way related to glacial-interglacial changes in climate.
Nss sulphates form Dimethyl sulphide (DMS) aerosols in the atmosphere (section 1.3.3). Consequently, increases in glacial ocean productivity may initiate another feedback mechanism, in addition to the productivity-CO2 connection. DMS represents the primary source of cloud condensation nuclei (CCN) in the marine atmosphere (Bates et al., 1987). Greater ocean productivity would thereby increase cloud cover, increasing the planetary albedo, and initiating further global cooling. Nevertheless, the magnitude of this feedback is uncertain. Indeed, increased cloud cover may enhance greenhouse warming of the lower atmosphere (section 2.7) and hence the reality of this mechanism must, for the while, remain in doubt.
Leave a Reply